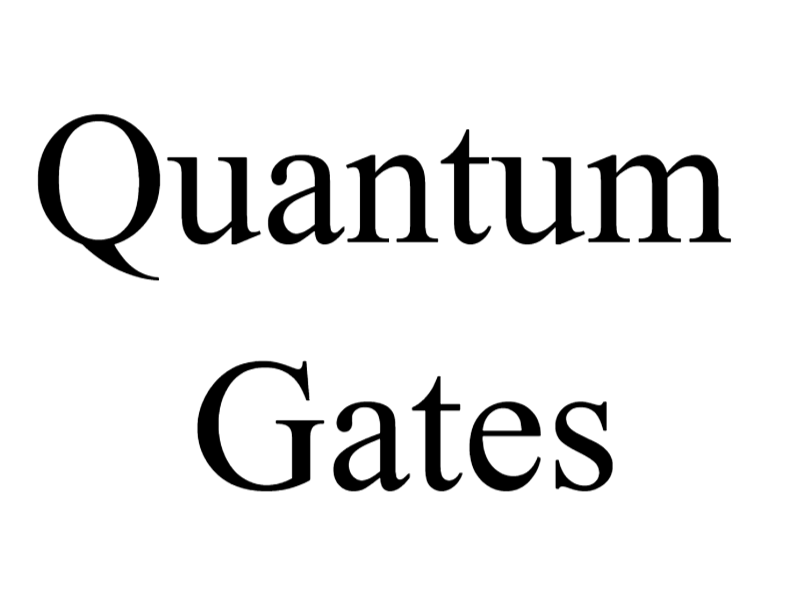
Quantum Gates: Building Blocks for Quantum Computation
Abstract:
Quantum gates are fundamental operations that manipulate quantum bits (qubits) and form the building blocks of quantum computation. This paper provides a comprehensive overview of quantum gates, including their mathematical representation, physical implementations, and applications in quantum information processing. We discuss common types of quantum gates, such as Pauli gates, Hadamard gate, and controlled gates, and explore their properties, quantum circuit representations, and the impact they have on quantum algorithms and quantum state manipulation.
Keywords: Quantum Gates, Quantum Computation, Qubits, Quantum Circuit, Quantum Algorithms.
Introduction:
Quantum gates are essential operations that allow for the manipulation of quantum bits (qubits) in quantum computation. They enable the encoding, manipulation, and measurement of quantum information. This paper aims to provide an in-depth understanding of quantum gates, including their mathematical representation, physical implementations, and applications in quantum information processing.
Mathematical Representation of Quantum Gates:
Quantum gates are mathematically represented as unitary operators acting on the Hilbert space of qubits. We introduce the matrix representation of quantum gates, which allows for the transformation of quantum states. Quantum gates are characterized by properties such as reversibility, unitarity, and their ability to preserve the norm of the quantum state.
Common Types of Quantum Gates:
We explore common types of quantum gates used in quantum computation. Pauli gates, including the Pauli-X, Pauli-Y, and Pauli-Z gates, rotate the quantum state around the X, Y, and Z axes of the Bloch sphere. The Hadamard gate introduces superposition and is a fundamental gate for creating entangled states. Controlled gates, such as the CNOT gate, enable entangling operations and conditional operations between qubits.
Quantum Circuit Representation:
Quantum gates are often represented using quantum circuits, which are graphical representations of sequences of gates acting on qubits. We discuss the circuit model of quantum computation, where gates are applied sequentially to the qubits. Quantum circuit diagrams provide an intuitive way to visualize and analyze quantum algorithms and operations.
Applications in Quantum Information Processing:
Quantum gates are crucial in quantum information processing tasks, including quantum algorithms, quantum error correction, and quantum simulation. They form the foundation for quantum algorithms such as Shor’s algorithm for factoring large numbers and Grover’s algorithm for unstructured search. Quantum gates are also vital in the implementation of quantum error correction codes and the simulation of quantum systems.
Physical Implementations of Quantum Gates:
We explore various physical implementations of quantum gates in different quantum platforms. These include superconducting circuits, trapped ions, topological qubits, and photonic systems. Each platform has unique advantages and challenges in realizing specific gate operations. We discuss the experimental progress and the potential for scalability in different physical systems.
Challenges and Future Perspectives:
The development of fault-tolerant quantum computers faces challenges such as decoherence, gate errors, and scalability. Error correction techniques and improving gate fidelities are crucial for mitigating errors and achieving reliable quantum computation. Continued research and technological advancements are necessary to overcome these challenges and pave the way for practical quantum computers.
Conclusion:
Quantum gates are the building blocks of quantum computation, enabling the manipulation of qubits and the implementation of quantum algorithms. Understanding their mathematical representation, physical implementations, and properties is vital for harnessing the power of quantum information processing. Quantum gates have significant implications in quantum algorithms, error correction, and quantum simulation. Continued research and technological progress will drive the development of practical quantum computers and revolutionize computation and information processing.
References:
- Nielsen, M. A., & Chuang, I. L. (2010). Quantum Computation and Quantum Information. Cambridge University Press.
- Preskill, J. (1998). Lecture Notes on Quantum Computation. California Institute of Technology.
- Devoret, M. H., & Schoelkopf, R. J. (2013). Superconducting circuits for quantum information: an outlook. Science, 339(6124), 1169-1174.
- Monroe, C., & Kim, J. (2013). Scaling the Ion Trap Quantum Processor. Science, 339(6124), 1164-1169.
- Awschalom, D. D., et al. (2018). Quantum Spintronics: Engineering and Manipulating Atom-Like Spins in Semiconductors. Science, 339(6124), 1174-1180.